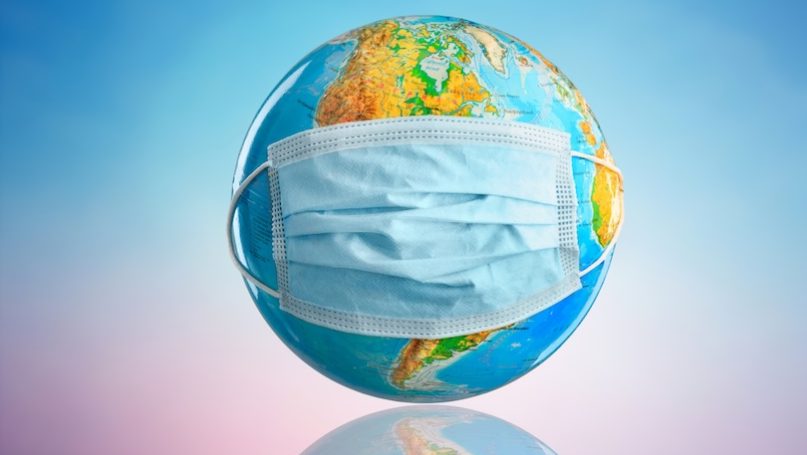
The COVID-19 pandemic reinforced the political value of disease surveillance mechanisms and tools. At the forefront of the response to this pandemic has been the extended use of genetic technologies to identify and map the changing nature of the SARS-CoV-2 virus. This was demonstrated most clearly in the identification of emerging new genetic variants from Alpha to Omicron. The knowledge regarding the genetic make-up of this virus was instrumental in the development of vaccines and diagnostic tests including reverse transcription polymerase chain reaction tests (RT-PCR) that revealed the burden of disease across certain populations (see Long 2023). This article investigates the impact that the use and spread of genetic technologies to combat this pandemic has had on the field of disease surveillance and the potential changes that it may bring in the future. In particular, it will focus on efforts to develop new global genomic surveillance strategies and the implications that this can have for the future of disease surveillance efforts within the One Health agenda.
The WHO and Global Disease Surveillance
Historically, disease surveillance efforts have led to the successful eradication of crippling diseases including smallpox. The negative public health impact of other diseases too has been minimised with the massive reduction in polio and measles in most parts of the globe. In achieving these objectives, infectious disease surveillance has three main goals: (1) to describe the current burden and epidemiology of disease, (2) to monitor trends, and (3) to identify outbreaks and new pathogens (Murray and Cohen 2017, 222). With regards to this third area, in particular, infectious disease surveillance is central to political efforts focused on detecting, responding to, and preventing outbreaks (Murray and Cohen 2017, 222).
Internationally, the WHO is responsible for determining the severity of disease outbreaks for the international community, and for guiding and coordinating the disease surveillance activities and the responsibilities of states. This set of powers and responsibilities is set out in the International Health Regulations (IHRs). These regulations have shifted over time in relation to the impact of certain disease outbreaks. In the wake of the severe acute respiratory syndrome (SARS) coronavirus outbreak from 2002–2004, the IHRs were revised in 2005 to allow the WHO to declare a Public Health Emergency of International Concern (PHEIC). This declaration can be made in relation to a number of events, including in ‘direct response to the emergence of novel pathogens and the re-emergence of known ones’ (Long 2023, 498).
It was also recognised that the IHRs, originally developed in 1969, were ‘no longer relevant to the health challenges the international community faced’ (Davies and Youde 2015, 11). The original IHRs, relied upon ‘passive surveillance mechanisms that focused on very specific diseases and offered no opportunity for non-state actors to play any role’ (Davies and Youde 2015, 11). The revised IHRs rested on states accepting a new norm under which they would engage in active disease surveillance out of a sense of obligation to their citizens and other countries (Davies and Youde 2015, 9). This sense of obligation went hand in hand with the new power of information communication technology to transmit notifications regarding disease spread and the recognition of the importance of respecting human rights in surveillance efforts (Davies and Youde 2015, 9). In this way, the revised IHRs also reflected the newfound understanding of sovereignty as responsibility that also emerged at the United Nations with the World Summit Outcome Document of 2005.
The WHO’s ability to declare a PHEIC brings into play a range of security measures including the invocation of national influenza pandemic preparedness plans, border closures, and quarantine measures that can significantly impact a country’s economy (WHO 2011a, 32-3). In a significant shift away from the previous regulations, the WHO can now declare a PHEIC and its associated recommendations without obtaining the permission of State Parties potentially harmed by such actions (Fidler 2005, 378). The determination and declaration of the existence of a PHEIC represented a new power for the WHO to securitise infectious disease outbreaks (see Long 2023). The WHO’s ability to determine the severity of a disease outbreak for the international community and securitise it via the declaration of a PHEIC is however dependent upon information that is fed into and shared with its surveillance networks (Long 2023, 501).
By far the largest surveillance network supported by the WHO is that focused on influenza. Created in 1947, the WHO’s Global Influenza Programme (GIP) sits at the core of this network with the aim of tracking and monitoring influenza outbreaks. To facilitate this, the GIP provides ‘Member States with strategic guidance, technical support, and the coordination of activities to help make their health systems better prepared against seasonal and pandemic influenza threats’ (Long 2023, 501). Information on these pathogens is collected, shared, and made intelligible via the national surveillance systems supported by the WHO (Long 2023, 498).
The WHO’s Global Influenza Surveillance and Response System (GISRS) is a global alert mechanism that is focused on identifying the emergence of influenza viruses with important features, including those that represent new seasonal variants and those with pandemic potential (WHO 2011b, 49). This potential is based on an assessment of its genetic code that determines its physical properties including levels of infectivity and transmissibility (Long 2023, 501-4). In this way, the GISRS provides early warning of genetic changes in influenza viruses circulating in the global population. This helps mitigate the consequences of potential pandemics and maintain the efficacy of seasonal influenza vaccines (Hay and McCauley 2018, 551). GISRS has within it a network of laboratories that collect and share information on viruses circulating across the globe. This includes WHO designated National Influenza Centres (NICs), WHO Collaborating Centres on influenza (WHO CCs), H5 Reference laboratories (H5RLs), and Essential Regulatory Laboratories (ERLs) that are coordinated by the GIP. The National Institute of Viral Disease Control and Prevention, part of the Chinese WHO CC in Beijing, would be the first laboratory to sequence the SARS-CoV-2 virus and share its genetic code online via the Global Initiative on Sharing All Influenza Data (GISAID) (Long 2023, 509-10).
Genomics and COVID-19
The COVID-19 pandemic revealed the immense power that genomics and genetic technologies can play in revealing the spread of this disease and in identifying the new variants that emerged. Genomics refer to the branch of biology that focuses on the ‘study of structure, function, mapping, and editing of the entire genome of an organism’ (Saravanan et al. 2022, 1). Further, it is also with the advent of next-generation sequencing platforms that genomics has been transformed from a discipline into a ‘technology that is commonly used in labs around the world to solve scientific problems’ (Saravanan et al. 2022, 1).
With regards to the COVID-19 pandemic, platforms such as this were used in a range of areas including in identifying the SARS-CoV-2 virus as the causative agent of COVID-19; understanding the characteristics of SARS-CoV-2 in terms of its infectivity; tracking SARS-CoV-2 variants and their spread; and developing diagnostic tools such as RT-PCR tests (Long 2023, 504-5), vaccines and therapeutics including antivirals (Saravanan et al. 2022, 2). Genomic surveillance information also enhanced risk evaluation regarding emerging variants and this shaped the public health measures implemented. The increased transmissibility of the Delta variant resulted in revised policy recommendations for indoor masking and the enhanced immune escape by Omicron resulted in updated vaccine recommendations (Ling-Hu et al. 2022, 12).
While the majority of mutations to viruses including SARS-CoV-2 are neutral and have little effect on the functional properties of the virus, certain mutations may be significant (Saravanan et al. 2022, 5). Changes in the genetic code of SARS-CoV-2 can result in different properties in the spike protein conferring selective advantages that lead to increased fitness thereby changing infectivity, transmissibility, host immunity responses, the effectiveness of vaccines, therapeutics, and other public health measures (Saravanan et al. 2022, 5). The WHO and its international networks tracked the evolution of the SARS-CoV-2 genome and spike protein generating variants of interest (VOI) and variants of concern (VOC) (Saravanan et al. 2022, 5). The analysis of SARS-CoV-2 genetic sequence data has also been used to calculate the expected number of new infections caused by an infectious individual (effective reproductive numbers (Rt)) and the transmission dynamics of SARS-CoV-2 that shaped public health measures, including regional lockdowns and travel restrictions (Saravanan et al. 2022, 5).
The spread of COVID-19 was declared a pandemic by the WHO on the 11th of March 2020. Following the sequencing of the virus it was found to be 96% identical to a coronavirus found in horseshoe bats (Saravanan et al. 2022, 3). In addition to unravelling the origin and evolution of SARS-CoV-2, genomic technologies have also revealed how the particular structure of this virus plays a key role in the disease-causing process. Understanding the genetic make-up of SARS-CoV-2 has allowed us to visualise the structures of the particular spike proteins that adorn the surface of this virus and that are reminiscent of a solar corona, hence its name (Long 2022, 138). Specifically, the ACE2 receptor on the surface of human cells is the target for the SARS-CoV-2 spike proteins (Jiang et al. 2020, 1134). By binding to and entering human cells at this site its reproduction and spread through the body is facilitated. Reverse genetics methods have also revealed that this binding site was shared with another virus from the same family – that of SARS-CoV-1 – the causative agent of SARS, noted above (Letko et al. 2020, 562). This knowledge has been utilised in the development of antivirals such as Bamlanivimab that bind to the specific and constant molecular site on the SARS-CoV-2 spike protein thus blocking it and preventing it from binding to the ACE2 receptor on the surface of human cells, preventing infection (Long 2022, 139; see also Long 2020).
The role of the spike protein in facilitating the entry of the SARS-CoV-2 virus into human cells during infection was also a key factor in the development of a range of vaccines. Different technological platforms were used in the development of vaccines including those that mobilised messenger ribonucleic acid (mRNA) to generate a protein that safely prompts an immune response (Mascellino et al. 2021, 3461). In the vaccines using this technology the genetic sequence coding for the spike proteins in the viral RNA was isolated into an mRNA sequence. Following entry into the human body via injection, the mRNA is synthesised by the ribosome in our cells resulting in the production of spike proteins via a process called translation (Mascellino et al. 2021, 3461). These proteins are then identified as targets by the immune system and the result is a response that produces antibodies that can recognise and neutralise the virus when it enters the body (Mascellino et al. 2021, 3461-2).
RT-PCR tests are another genetic technology that served as a key surveillance and diagnostic tool during the pandemic. This tool helps identify if someone is infected with a particular virus and thus make measurable and visible infection rates across a population (Long 2023, 504). The understanding of the full genome sequence of a virus is important in the development of RT-PCR tests as this technology detects parts of the genome and particular genes of the virus in question (Long 2023, 505). Not only are genetic sequences necessary for the development of these tests but enough genomes of a new viral variant must be sequenced – not only to verify its public health relevance, but also to confirm the presence and high prevalence of genetic changes that allow specific tests to be developed (Brito, et al. 2022, 4). Specifically, this test amplifies a specific part of the DNA strand creating many copies that can serve as a clear and visible identifier of infection (Long 2023, 505). This amplification process is known as polymerase chain reaction.
Genetic sequencing technologies were also used to an unprecedented degree during the COVID-19 pandemic with over 14 million cases sequenced and a huge number of countries around the world producing their own genomic data despite the prevalence of socioeconomic inequalities impacting the sequencing of genomes and the speed with which this data was shared (Hill et al. 2023, 863; Brito, et al. 2022; see also Papamichail 2023). The urgency of the pandemic was also leveraged to rapidly build sequencing capacity, particularly in Africa and the Caribbean with substantial investment during 2021 enabling the African CDC to set up or support ten African sequencing hubs (Hill et al. 2023, 863). This effort raised the number of African countries that had in-country SARS-CoV-2 sequencing capacity from 20 of 55 (36%) in April 2020 to 39 (71%) by mid-2022 (Hill et al. 2023, 863). A similar development occurred in the Caribbean with there being limited local capacity for viral genomic surveillance prior to the pandemic. In late 2020, ONT MinION, a small portable sequencing device, was utilised at The University of the West Indies in Trinidad and Tobago providing capacity for 17 member states of the Caribbean Public Health Agency (CARPHA) (Hill et al. 2023, 864). In December 2021, CARPHA developed its own in-house capacity and at least 8 of the 30 Caribbean islands have access to sequencing technology and produced their own SARS-CoV-2 sequences (Hill et al. 2023, 864).
The COVID-19 pandemic also represented the successful development and strengthening of existing regional sequencing centres. This included centres in South Africa, Nigeria and Senegal (Hill et al. 2023, 864). Other countries such as the UK made use of their long-standing academic and public health partnerships to pivot clinical and academic laboratories to rapidly sequence SARS-CoV-2 via the COVID-19 Genomics UK (COG-UK) consortium (Hill et al. 2023, 864). A number of other countries around the world set up genomics consortium and sequencing initiatives including Canada, Denmark, Germany, India and Ireland. Genomic surveillance was also supported via the creation of a number of global networks including the Advancing Real-Time Infection Control (ARTIC) network’s Real-Time Molecular Epidemiology For Outbreak Response, COVID-19 High Performance Computing (HPC) Consortium, Public Health Alliance for Genomic Epidemiology (PHA4GE) and The COVID-19 host genetics initiative (Saravanan et al. 2022, 4).
The sequence data of SARS-COV-2 genomes from these local and global efforts are now shared through open access repositories such as GISAID. This repository was initially developed for the rapid international exchange of all influenza viral genomes and related clinical data and it was expanded to include SARS-CoV-2 genomic data (Saravanan et al. 2022, 4). Already, by December 2021, ‘more than 5.7 million SARS-CoV-2 genome sequences from nearly 200 countries around the world were shared on the GISAID database’ (Saravanan et al. 2022, 4). To date, 219 countries and territories have shared 16,843,380 viral genome sequences from human cases of COVID-19 via GISAID since the 10th of January 2020 (GISAID 2025).
Another advancement during the pandemic was the development of a lineage classification system, providing a relatively universal, high-resolution picture of the genetic diversity of this virus (Hill et al. 2023, 864). Wastewater genomic surveillance, despite limitations, also emerged as a useful tool in monitoring the levels of a virus in a given community when a ‘sufficient amount is known about how case counts correspond to the virus concentration in sewage’ (Hill et al. 2023, 867). The rapid uptake and expansion of the use of genomic technologies during the pandemic will go on to shape the future of disease surveillance and our insight into the relationship between ourselves and the natural world in the prevention of outbreaks.
The Future of Disease Surveillance
Following the relative resolution of the COVID-19 pandemic there has been a call to build on the finance and training invested in addressing this threat and to direct this into a rapid response system to combat endemic viruses and prepare for the next pandemic (Hill et al. 2023, 865). The expanded sequencing capacity developed provides an opportunity to create global genomic surveillance systems for other medically important viruses (Hill et al. 2023, 865). The pipelines dedicated to the sequencing of SARS-CoV-2 viruses can be diverted, rather than closed, to sustain funding and maintain access to diagnostic samples, employment of trained personnel, equipment functionality, supply stocks, database management and protocol continuity (Hill et al. 2023, 865).
The diversion of these resources should take place in tandem with the promotion of the value of routinely sequencing medically important endemic viruses as a standard component of surveillance (Hill et al. 2023, 866). This could enhance forecasting and create the infrastructure to rapidly and efficiently respond to outbreaks benefiting public health (Hill et al. 2023, 866). A global virus genomic surveillance network can offer up a number of insights including baseline genetic diversity to help design intervention strategies; track the effectiveness of vaccines or other control measures at the genotype level; detect the emergence of new variants as an early warning for increased cases; and monitor changes in transmission routes and the global spread of pathogens (Hill et al. 2023, 870).
A global virus genomic surveillance network utilising pathogen genomic sequencing can also help contribute to the One Health agenda focused on tackling emerging disease threats. This agenda recognizes that the health of people is closely connected to the health of animals and our shared wider natural environment (Hill et al. 2024, 1; see Long 2022, 121-34). The effects of globalisation including increased travel, trade, intensive farming practices and climate change, may amplify risks associated with the emergence of zoonotic pathogens in humans (Hill et al. 2024, 1). In response, efforts have been developed to bring together experts from a range of sectors – including human, animal and plant health and the environment – in order to build a detection and response infrastructure that emphasizes the sharing of information and the coordination of actions across these sectors (Hill et al. 2024, 1).
The inclusion of laboratory diagnostic and epidemiological data covering humans, domestic animals, and wildlife alongside environmental and biodiversity data, promises to provide a more holistic picture of how pathogens spread and develop within a One Health framework (Oltean, et al. 2025, 2). The analysis of pathogen genomic data ‘allows for the assessment of transmission dynamics at the human-animal-environment interface’ (Oltean, et al. 2025, 2). This form of analysis can also be applied across a range of different pathogens including bacterial, viral and fungal (Oltean, et al. 2025, 2). Within this, wastewater surveillance may be a vital source of health-related data allowing for the simultaneous detection of chemicals, pathogens and other microorganisms that can facilitate a better understanding of how diseases are transmitted in the environment (Hill et al. 2024, 2). Overall, the implementation of a global and integrated genomic surveillance system can support early outbreak detection and provide an improved understanding of pathogen reservoirs, evolution, and modes of transmission (Hill et al. 2024, 2). Such insights will support a more holistic understanding of threats to global health within the One Health framework and provide political efforts with more proactive prevention measures.
References
Brito, Anderson F. et al. 2022. “Global disparities in SARS-CoV-2 genomic surveillance.” Nature Communications 13(1): 1-13.
Davies, Sara E and Jeremy R. Youde. 2017. “Surveillance, Response and Responsibilities in the 2005 International Health Regulations.” In The Politics of Surveillance and Response to Disease Outbreaks, edited by Sara E. Davies and Jeremy R. Youde, 9-22. Abingdon, Routledge.
Fidler, David P. 2005. “From international sanitary conventions to global health security: The new international health regulations.” Chinese Journal of International Law 4(2): 325–392.
GISAID. 2025. “Global Submission Tracker.” Accessed April 15, 2025. https://gisaid.org/submission-tracker-global/
Hay, Alan J. and John W. McCauley. 2018. “The WHO global influenza surveillance and response system (GISRS): A future perspective.” Influenza and Other Respiratory Viruses 12: 551-557.
Hill, Richard et al. 2024. “Realising a global One Health disease surveillance approach: insights from wastewater and beyond.” Nature Communications 15(1): 1-6.
Hill, Verity, George Githinji, Chantal B.F. Vogels, Ana I. Bento, Chrispin Chaguza, Christine V.F. Carrington and Nathan D. Grubaugh. 2023. “Toward a global virus genomic surveillance network.” Cell Host & Microbe 31: 861-873.
Jiang, Shibo, Xiujuan Zhang, Yang Yang, Peter J. Hotez & Lanying Du. 2020. “Neutralizing antibodies for the treatment of COVID-19.” Nature Biomedical Engineering 4: 1134–1139.
Letko, Michael, Andrea Marzi and Vincent Munster. 2020. “Functional assessment of cell entry and receptor usage for SARS-CoV-2 and other lineage B betacoronaviruses.” Nature Microbiology 5: 562–569.
Ling-Hu, Ted, Estefany Rios-Guzman, Ramon Lorenzo-Redondo, Egon A. Ozer and Judd F. Hultquist. 2022. “Challenges and Opportunities for Global Genomic Surveillance Strategies in the COVID-19 Era.” Viruses 14(11): 1-21.
Long, Christopher. 2020. “Challenging contingency: Viruses and the nature of molecular life.” Security Dialogue 51(4): 323–339.
Long, Christopher. 2022. The Molecularisation of Security: Medical Countermeasures, Stockpiling and the Governance of Biological Threat. Abingdon, Routledge.
Long, Christopher. 2023. “Securitising infectious disease outbreaks: The WHO and the visualisation of molecular life.” European Journal of International Security 8: 493–512.
Mascellino, Maria Teresa, Federica Di Timoteo, Massimiliano De Angelis & Alessandra Oliva. 2021. “Overview of the Main Anti-SARS-CoV-2 Vaccines: Mechanism of Action, Efficacy and Safety.” Infection and Drug Resistance 14: 3459–3476.
Murray, Jillian and Adam L Cohen. 2017. “Infectious Disease Surveillance.” International Encyclopedia of Public Health 4: 222-229.
Oltean, Hanna N et al. 2025. “Developing a one health data integration framework focused on real-time pathogen surveillance and applied genomic epidemiology.” One Health Outlook 7(9): 1-14.
Papamichail, Andreas. 2023. “Reinscribing global hierarchies: COVID-19, racial capitalism and the liberal international order.” International Affairs 99(4): 1673–1691.
Saravanan, K. A. et al. 2022. “Role of genomics in combating COVID-19 pandemic.” Gene 823: 1-11.
WHO. 2011a. Strengthening Response to Pandemics and Other Public Health Emergencies: Report of the Review Committee on the Functioning of the International Health Regulations (2005) and on Pandemic Influenza (H1N1) 2009. Geneva: WHO.
WHO. 2011b. Pandemic Influenza Preparedness Framework for the Sharing of Influenza Viruses and Access to Vaccines and Other Benefits. Geneva: WHO.
Further Reading on E-International Relations
- Covid-19: Learning the Hard Way
- Global Health Diplomacy and the Security of Nations Beyond COVID-19
- The International Political Economy of Health: The Covid-19 Vaccine Distribution
- The Appeals and the Limits of Digital Education in the Post-Covid Era
- Why the Pandemic Treaty Must Reclaim Human Rights and Equity
- A Blessing in Disguise: UAE’s Possible Scenarios for a Post-COVID-19 World